Who hasn’t enjoyed heat from the sun? Doing so represents a direct energetic transfer—via radiation—from the sun’s hot surface to your skin. One square meter can catch about 1000 W, which is comparable to the output of a portable space heater. A dark surface can capture the energy at nearly 100% efficiency, beating (heating?) the pants off of solar photovoltaic (PV) capture efficiency, for instance. We have already seen that solar PV qualifies as a super-abundant resource, requiring panels covering only about 0.5% of land to meet our entire energy demand (still huge, granted). So direct thermal energy from the sun, gathered more efficiently than what PV can do, is automatically in the abundant club. Let’s evaluate some of the practical issues surrounding solar thermal: either for home heating or for the production of electricity.
Heat as Something Useful
In physics classes, I often catch myself repeating the mantra that heat is a disordered, useless state of energy that is generically the endpoint of an energy flow process. For example, the energy allocated to the fast-spinning wheel of an upside-down bicycle will slowly drain away as the wheel stirs the air, makes sound, and suffers friction at the bearing. Every one of these energy paths results in heat, until 100% of the invested energy is dissipated and the room is a tad warmer as a result. We will never reassemble the lost energy into useful form, once entropy has claimed it. All of this is true enough, but I feel very awkward uttering the words that heat is the graveyard of energy flow, and must place an asterisk on the statement.
The asterisk is that the overwhelming majority of our societal energy consumption makes use of heat—over 90% in the U.S.! So heat does not deserve the bad rap as a worthless waste product. Rather, heat runs our world! Sometimes we just want the heat directly, via: natural gas for furnaces, hot water, and cooking; heating oil for the home; and gas and coal for industrial process heat. This accounts for 20% of our total energy demand, leaving about two-thirds of our total energy consumption in the form of heat that powers heat engines for electricity production, transportation, and machinery. In short, all the energy we get from fossil fuels, nuclear, and biomass derives from heat. That’s hardly useless!
Radiant Heat
The Sun transmits its energy to Earth across the emptiness of space via radiation. Each square meter of surface at a temperature, T, emits radiation at a rate of σT4, where T is expressed in Kelvin (important!) and σ = 5.67×10−8 W/m²/K4. This constant is easy to remember via the sequence 5-6-7-8. Ignoring for now the subtleties of greenhouse gases, the surface of Earth—typically at 288 K—emits 390 W/m². The Sun, on the other hand, at 5800 K, emits 64 MW per square meter!
Summing over the area of the spherical Sun, at 109 times the radius of Earth, we find the total radiant power of the Sun to be a whopping 3.9×1026 W. Now that’s a light bulb! The Sun’s radiant energy spreads into all directions, creating a sphere of light. At the distance of the Earth, that sphere has an area of 4πr² ≈ 2.8×1023 m², where r is the mean Earth-Sun distance. Dividing these huge figures, we find that the radiant intensity at Earth is 1370 W/m²—which I hope will be a familiar number by now for Do the Math readers.
We can also turn the σT4 relation on its head and say that a patch of full sun (at the ground) receiving 1000 W/m² corresponds to a radiant temperature of 364 K, or a blistering 91°C. This means that a black panel in full sun could get this hot if no paths other than radiation were available for cooling the panel. We would then say that the panel is in radiative equilibrium with the Sun. But air can carry away heat by convection. The self-convection of a hot, flat plate will be about 10 W/m² per degree of difference between the panel and the surrounding air. Requiring the sum of radiative and convective losses to add up to the input power of 1000 W/m² yields a solution of about 55°C (328 K; 131°F) if the surrounding air is at 20°C. This assumes that the plate has no heat paths available through the (insulated) back side. If, on the other hand, it is a thin panel allowing convection on both sides, it will be cooler—although the “heat rises” phenomenon will suppress heat flow on the back side relative to the front, if the plate is indeed level. Just for fun, if we get an additional 5 W/m²/K of convective loss off the back, the equilibrium temperature drops to 47°C (117°F). It all seems reasonable.
Passive Solar: Putting Heat to Use
The simplest way to replace fossil fuel energy with solar energy is called a window. A single uncoated piece of glass will transmit 92% of visible light (the rest reflected) when light comes straight in (down to 75% at a 20° grazing incidence, 60% at 10° grazing). The glass is opaque to ultraviolet light and mid- to far-infrared (IR) light, but lets over 95% of the unreflected incident solar spectrum pass.
Considering that windows in houses/buildings tend to be vertical, we can evaluate the energy input through windows, taking transmission loss, reflection loss, and angular foreshortening into effect. Because the Sun is higher in the sky in the summer, the window appears foreshortened to the direct sunlight, and also reflects more. So a south-facing window automatically admits more heat in the winter than in the summer, with no adjustment. Putting an overhang over the window—ideally with some vertical space between the window and overhang—can eliminate the summer noon-time contribution entirely. The figure below illustrates the fraction of incident direct-sun energy (think 1000 W/m²) admitted by the window. Vertical reference lines indicate the noon-time elevation of the sun at a latitude of 40° for the winter and summer solstices. The noon-day sun will be somewhere between these values all year. Adjustment to other latitudes involves a simple shift of the dashed lines by the latitude difference.
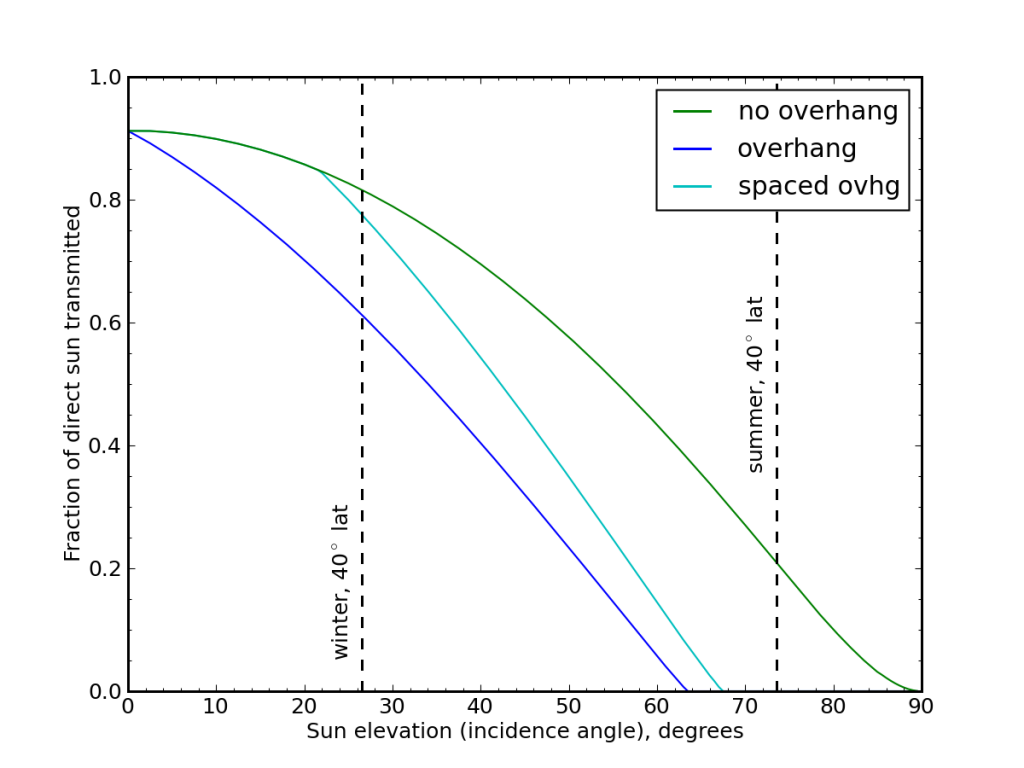
Fraction of incident direct energy (perpendicular to rays) making it through a vertical window. The overhang extends an amount that is half the window height, and optionally is spaced 0.2 window-height-units above the top of the window.
So it is not a stretch to admit energy in excess of 500 W/m² into your home in winter sun. You can stack up the equivalent of a dozen or so space heaters pretty quickly.
Drab Winter?
Sounds great, but winters are not always the sunniest of times. However, it’s not as bad as you might fear. Every photon of visible light that makes it through your window—even if coming from a drab gray cloud—deposits the same amount of heat no mater how convoluted its path from the Sun. Indeed, a measurement campaign in my home has revealed that the attic gets surprisingly warmer (10°C, or 18°F) than the ambient air even on a day of heavy clouds when my solar PV system only caught one-quarter of the usual amount of light. So we can use the NREL database for a flat plate collector (in this case, a window) oriented south at a 90° tilt to represent the amount of energy a window would grab. The following table indicates the equivalent number of full-sun hours per day during the heating months for Seattle, WA (on the poor end), St. Louis MO (a representative U.S. average solar city), and San Diego (my home).
City | Oct. | Nov. | Dec. | Jan. | Feb. | Mar. | Apr. |
Seattle, window | 2.7 | 1.7 | 1.3 | 1.5 | 2.2 | 2.8 | 3.0 |
Seattle, full sun | 2.3 | 1.1 | 0.9 | 1.0 | 1.7 | 2.5 | 3.1 |
St. Louis, window | 3.8 | 3.2 | 3.0 | 3.5 | 3.7 | 3.4 | 3.1 |
St. Louis, full sun | 4.1 | 2.9 | 2.4 | 2.9 | 3.2 | 3.6 | 4.3 |
San Diego, window | 4.4 | 4.6 | 4.5 | 4.5 | 4.3 | 3.9 | 3.2 |
San Diego, full sun | 5.3 | 4.9 | 4.5 | 4.5 | 4.8 | 5.1 | 5.8 |
The table also includes the number of equivalent full-sun hours per day a 2-axis concentrating system would recover, which is a good proxy for the average daily number of hours of direct sun. The window can often get more energy than is present in full sun due to the diffuse gain, which is the case for six out of the seven months for Seattle in the table above.
If a house had four large windows facing south, each 2 m wide and 3 m tall, a typical Seattle day in December would deposit 900 W/m² (from earlier graph, low elevation sun) times 24 m², or about 22 kW of power for about 1.3 hours. This amounts to 28 kWh of energy (corresponding to about 1 Therm of natural gas energy).
To make this amount of heat work, the house must be extraordinarily well insulated, use fancy windows, and be draft-free—using a heat recovery ventilator. But such feats can be accomplished in passive home design, even in climates that would appear to be completely hostile to the notion of passive solar heating.
It is also often advantageous to have several days’ worth of thermal storage in the home to average out the sunny and cloudy days. A dark, massive rock or brick wall can do the job—preferentially opposite the huge south-facing windows to directly soak up the solar input. At a specific heat capacity of 1000 J/kg/K and a density of 3000 kg/m³, a rock wall 0.5 m thick and matching our 24 m² window footprint will see a temperature rise of about 2°C for every hour of sunlight poring onto it. A good sunny day pumping five hours of solar energy into the mass would raise its temperature 10°C, so that lazy air pulling off heat at 2 W/m²/K would initially pump out 2400 W of power after the sun is down (assuming the back of the wall is insulated), and provide about 2 days of heat with no additional input.
Of course a number of engineering challenges surround clever passive solar thermal design, and I should pull away before the post gets bogged down (too late, you say?). Perhaps I will return to the topic later. For now, it is worth understanding that the amount of solar radiation incident on a house can be sufficient to provide heating even in unfavorable climates. I should add one caveat: that passive heating may be sufficient 90% of the time, requiring either backup heat or—preferably—flexibility in dealing with a colder house the other 10% of the time.
Hot Water
Using the sun to heat water is a very similar concept. We saw that a flat black plate in the sun can get pretty toasty. In practice, flat panel collectors can hang onto about 60% of the incident solar energy, transferring this to the water. Heat paths via radiation through the glass on the front, convection of air within the panel, and conduction through the back and mounting frame all contribute to loss. For radiative loss, radiation from the black panel is intercepted by the glass (thermal IR is not transmitted by glass), warming it up. This can then radiate both skyward and back to the absorber. A second piece of glass (double-pane) can cut down radiation losses, by returning approximately half of what would otherwise have been lost off the front panel. Some fancy units evacuate air to minimize convective loss, and the backs can be insulated to reduce loss. Given all these thermal leaks, holding on to 60% of the incident energy is pretty impressive.
Let’s assume your household requires 300 liters of hot water each day—the equivalent of four “long” 10 minute showers at a healthy flow of 8 liters per minute. This, by the way, is far more than I believe is really necessary for a household—even if it is typical. If the water comes in at 10°C, and is heated to 60°C, then we need to supply 15,000 kcal of energy—following the definition of the kilocalorie. Considering 60% efficiency and allowing for some daily loss in storage, we need to provide 30,000 kcal of solar input each day, amounting to 35 kWh of energy. As it turns out, tilting a panel to 54° in St. Louis gives at least 3.5 hours of full-sun-equivalent (1000 W/m²) even in December, so that we need 10 m² of panels (a bedroom’s size).
Solar Thermal Electricity
The relatively low temperatures achieved by flat panels in the sun do not encourage exploitation in the form of heat engines for making electricity. But we can fix this through the simple act of concentration. No—not simply thinking really hard about it. Much like a magnifying glass can be used to burn paper, any piling-up of solar flux can elevate the temperature. I have personally melted pennies, boiled water, and turned sand into glass with a large hand-held Fresnel lens. Even a bunch of flat mirrors directing sunlight onto a common spot can create formidably high temperatures.
Concentration is expressed as a ratio, so if I take a circular magnifying glass 100 mm in diameter that makes an image of the sun 1 mm across, the concentration factor is 10,000 (the ratio of areas). Using our radiative relation, the resulting 10 MW/m² corresponds to a temperature of 3600 K! That kind of temperature will melt any metal, if you put the concentrated light onto a fleck of metal smaller than the bright spot. Typical boilers in power plants produce a hot temperature of about 1000 K. Achieving a comparable temperature via solar input requires a concentration in excess of 60.
One downside is that concentration implies tracking, which adds to complexity. Two-dimensional concentration—like a magnifying glass—requires two-axis control to keep the hotspot on the small target. One-dimensional concentration—such as a parabolic trough—only requires tracking along one axis. The concentration ratio of a 1-D concentrator is roughly the square-root of the 2-D variety, but that’s okay if we only need concentration ratios around 100 or so. One-dimensional concentration is also far more forgiving of imperfections in the reflector shape (can be made more cheaply).
Another downside of concentration is that it requires real direct sunlight to work. Can you see a sharp shadow on the ground? If not, concentration is effectively dead. In essence, the concentrator is forming an image of the sun—sometimes a stretched-out linear image in the case of trough collectors. Forming images of clouds onto the collector will not get it very excited. It needs the real thing. Comparing the effective yield for tracking configurations at different sites gives some sense for how some places are differently advantaged to exploit solar thermal. In general, desert areas do very well.
City | Flat Panel at Lat. | 1-axis, N–S | 2-axis |
Seattle, WA | 1.4/3.7/5.7 | 0.4/2.5/5.2 | 0.9/2.9/5.5 |
St. Louis, MO | 3.1/4.8/5.9 | 1.5/3.5/5.3 | 2.4/4.1/5.5 |
San Diego, CA | 4.6/5.7/6.5 | 3.8/4.1/4.9 | 4.5/5.3/6.3 |
Dagget, CA | 5.2/6.6/7.4 | 3.5/6.6/9.6 | 5.4/7.5/9.7 |
The table above gives average daily yields (kWh/m²/day, or equivalent hours at 1000 W/m²) for three types of solar collection in four locations, each entry giving worst-month/yearly-average/best-month values. The first is for a flat plate tilted to the site latitude (appropriate for PV or hot water), followed by 1-axis concentration tilting along a N-S axis, and finally a 2-axis concentration configuration. Solar thermal makes the most sense in areas where more energy will be collected than with PV panels—but this is not a rigorous criterion, since solar thermal offers some advantages over PV, as we’ll discuss in a bit. In the table above, only Dagget, California—in the Mojave desert— has concentration beating flat-panel PV for total energy. Other desert cities in the Southwestern U.S. likewise are favorable toward solar thermal electricity. But it’s definitely a location-dependent technology.
Solar Thermal Schemes
Schemes abound: 1) power towers where an array of individually-steered flat mirrors are angled to put sunlight at the top of a tower in the middle of the array; 2) satellite-dish-looking segmented bowls with a heat engine at the focus; 3) parabolic trough arrays with a hot-oil-carrying pipe running down the focus; 4) and others topologies, I am sure.
Taking the simple parabolic trough as an example, about 70% of the incident energy makes it into the 400°C fluid running within the central pipe. Heat carried by the oil makes steam to turn turbines, in the traditional power plant sense. The efficiency of the power plant portion is in the usual ballpark of 30%. These two factors alone produce 20% efficiency, but other losses tend to push it down to 15% or so. The troughs are typically oriented north-south, with daily tracking (e.g., pivot about the hot pipe). Self-shadowing becomes an issue, mitigated by providing ample room between collectors. If you want to track the sun as low as 15 degrees elevation with no shadowing, for instance, only one-fourth of the land area is utilized. East-west orientations are also possible, performing less well year-round, but more uniformly throughout the year.
Parabolic troughs are pretty neat, I think, for a variety of reasons. First, the parabolic shape accomplishes focus independent of the slant angle of the light in the direction along the axis: mathematical perfection no matter the angle. This leads to the second serious advantage—already discussed—of single-axis tracking along a north-south axis. The ability to transport the heat along the axis using a fluid/pipe is unique to this design, making it convenient to schlep the heat around where you want it. Finally, because the shiny material only needs to be bent in one direction (far easier than a bowl-shape), the reflectors are relatively inexpensive to make.
Evaluating a realized example, the Nevada Solar One plant has a 64 MW nominal capacity, generating 134 million kilowatt-hours of energy per year. Dividing these two implies about 2100 hours of full-power operation per year, for a duty cycle of 24%, or 5.7 hours per day. The NREL database for Las Vegas expects a north-south single-axis tracker to get an average of 6.2 hours per day horizon-to-horizon. So not too far off. The plant cost $266 million to build, amounting to $4.15 per Watt. Pretty similar to installed solar PV. The plant occupies about 1.6 km² of land, computing to 40 W/m² at nominal full power. This is 4% of the incident 1000 W/m² (at the height of summer), which is pretty close to what we would guess for a 15% efficient collector occupying 25% of the land area. I love it when the numbers make sense!
A Storage Boon
One serious perk to solar thermal—not yet exploited as fully as it might be—is thermal storage. Make hay when the sun shines, and squirrel it away for overnight use. All solar thermal plants have short-term immunity from intermittency due simply to the thermal mass in the system. Solar thermal plants are designed with varying degrees of storage, many just aiming for several hours to better follow the peak demand curve into the evening. But as renewables gain dominance over fossil fuels (as I’m hoping they do), storage will become increasingly important. To my mind, the ratio of storage to collection is pretty straightforward to change (i.e., bigger vat of hot fluid), so that in principle solar thermal plants could achieve days of storage with little added complexity. We can’t say this about PV or wind. And storage efficiency for a large container grows linearly with the tank’s dimension, since it the energy contained scales like volume, while thermal loss paths tend to scale with area.
One of the Winners
We looked at three categories of using heat from the Sun: passive home heating, hot water, and solar thermal electricity. Virtually anything involving direct use of solar energy—as opposed to hydroelectric, wind, waves, etc. as secondary and tertiary derivatives of solar input—is bound to end up on the abundant side of the story. And so it is with these three, although perhaps given that the first two are confined to the meager area represented by rooftops and/or windows—rather than the entire land area—they should more fairly be stashed in the “potent” box.
Solar thermal electricity definitely joins the camp as an abundant resource. Some of the other abundant resources described to date (nuclear breeders, geothermal depletion, and more to come) present technical hurdles or other practical barriers that diminish my excitement for them. I won’t claim that solar thermal electricity has no difficulties (reflectors get dusty/abraded by desert sands, for instance). But it’s pretty low-tech, utilizes over a century of experience in running heat engines, allows storage to be an integral part of the design, and is super-abundant on the scale of things. All the same, we have found yet another viable way to make electricity, doing little to directly address a liquid fuels shortage.
The low-tech nature of solar thermal makes it especially robust in tough times. I can imagine personally designing and building a passive solar home, flat-plate thermal collectors for hot water, and even a parabolic trough to create steam. I can’t say the same about a PV panel, a nuclear reactor, or geothermal wells kilometers deep. It gets my vote.
We’ll see nuclear fusion next week. Sound familiar?
Views: 19840
The only thing u dont note is Snow coverage, in a great portion of the country you would have to provide heating of a houses roof to melt the snow covering the roof. That is snow is on the ground from about December ~ March and to keep a roof completely clear u’d need to melt at between 40 inches of snow to well over 200 INCHES (think Michigan UP). Thats if u were to melt just the snowFALL, wind is going to blow enough snow on your roof each day to effectually remove this as a viable option in large swatches of the country as you end up spending more energy removing snow than u gain via heating.
But after heavy snowfall, home owners usually shovel the snow off their roofs anyway to prevent roof avalanches and possible structural problems. So no need to melt through 200 inches of snow! (By the way, that would be five meters. Such an amount of snow could never build up on a slanted roof, because it would slide off way before it reached that height, possibly burying you or passers-by in the process. Flat roofs on the other hand would collapse if you allowed five meters of snow to build up!)
This leaves snow drift as a possible problem, but for the snow to be blown up onto your roof (around eight meters above ground level for average family homes, and much higher for apartment buildings) it would take a combination of light powder snow and very strong winds or even a storm. These factors assure that only a very thin cover can build up on your roof (the wind blows snow up onto the roof, while also blowing snow away from the roof at the same time). This thin cover will melt quickly when the sun returns. Also, remember that the solar collector panels are black and heat up very quickly, which means they will be the first snow-free parts of your roof.
By the way, I’m not just speculating here, I have first-hand experience with both heavy snowfall and passive solar heating – I grew up in the Alps.
200 inches of snow doesn’t fall in 1 day, it falls over the course of many months. To keep it clear you’d still have to melt ALL the snow on the roof. It may not be 200 inches that your melting through as u may have 2-3 snow falls a year where u scrape u 20 inches or so but on average not EVERY snowfall is scraped off of roofs. Where i grew up we got on average 100 or so inches and u NEVER went onto the roof to remove snow.
Either way from my experience with snow (midwest usa 20+years) u still have the issue of wind blowing 1/2 inch or so of snow enough to block your passive heating onto the lower part of your roof ( they start around 10 feet not 8 meters) and its going to be a constant issue with ALL types of snow as even a light breeze can blow snow out of the trees and flat top black tarred buildings end up with around 2 inches of snow on them only hours after being scraped.
Ether way that’s just notional based on my experience I’d be curious how much effort (daily or weekly?) ends up being required to keep this clear of snow. I’d imagine a wide scale implementation of this on house tops would need to have ZERO upkeep as compared to a regular roof to be widely accpeted.
The idea for large “windscreen wipers” for a roof just appeared in my mind.
Or scale up one of these:
http://www.amazon.co.uk/Betterware-708-Telescopic-Conservatory-Cleaner/dp/B000K4X7BK
At least snow cover won’t impact the notional 4-window example given. If there is any solar benefit to the higher latitudes where snow is likely to occur, it may be that the steep tilt of flat solar collection surfaces will not hold snow for long.
Snow is fairly translucent so usually PV collectors heat up enough to make even 10cm of snow cover slight down the sloped surface. This is my experience from a PV installation in Germany. The story might be different if you get a meter of snow in one of those blizzards in North America.
Its all very well putting big solar power plants in deserts, but most people don’t live in deserts, or even very near them. Your personal geography is unusual in that you don’t have to cross a sea, a large distance, or any serious political boundaries to get from where you are to desert terrain.
Do you think there is going to have to be a mass shift in human populations in the future, or do you think we will be able to cope with the technical/logistic/political issues of having power stations so far from power users?
It’s true that I live close to ample solar resources. And while solar thermal works best in deserts, it’s not a total wash in most places in the U.S., actually. It’s just disadvantaged enough that you’re unlikely to see installations crop up in the current climate.
Perhaps, but in the UK (and most of the EU) it isn’t so easy. There are some good spots in southern Europe (Spain has one of those big solar power towers) but most large scale plans look to the Sahara desert, which is technically and politically difficult.
electricity is actually quite easy to transport over large distances, much easier than heat or fossil fuels. 1000s of km with still tolerable losses when using AC, 10000s if you use high voltage DC. It’s really not the problem it’s cracked up to be in the media all the time. The existing energy monopolies just don’t want to invest in infrastructure, instead milking the existing (and fully depreciated since decades) infrastructure for every cent they can get out.
Excellent analysis. Now I can stop badgering you to post on this subject!
On the note of low-tech, robust solar heating panels, visit http://www.builditsolar.com/ for plenty of ideas on how to do just what you were saying.
The fusion post is always a week away. I work in plasma physics / fusion science so I’m looking forward to reading what you have to say on the matter.
The fusion post is always a week away…
kinda like fusion power itself, which has been 20 years away for the last 30 years or so…
You guys are catching on to my little joke. Don’t tell anyone else…
But you covered fusion this week!
I guess given that PV, solar thermal, wind, hydroelectric, biofuel are all solar, I’ve indirectly covered fusion lots of times now…
My only comment would be that this talks about article talks about efficiencies of solar which when abundant is somewhat meaningless, what about the economic costs. I know other articles haven’t gone into that in too much detail, but I guess that is a comment on the series then
I’d also be interested in what thoughts you had on the solar updraft towers…
That’s probably outside the scope of this website. The cost of solar power, for right now, is mostly determined by what kind of financing deals they can get. It comes down to the bank’s personal opinions as much as anything, and seems to vary widely from one location to another.
http://www.eurotrib.com/story/2009/5/1/174635/6513 is my go-to source on the subject.
A natural gas plant’s costs are mostly fuel costs, with a bit of capital, while renewable power is mostly capital cost; also one can shut down waiting for high prices, the other sells at whatever market price is. So even if they can produce electricity at the same average cost, they have very different relations to finance and pricing, and renewable is far more sensitive to interest rates.
And who has the lowest interest rates? Government, presumably followed by big business, and small entrepreneurs last. The very decision to “leave it up to the markets” builds in a bias against “alternative energy”, and renewables could become more economical simply by having government build them.
I can’t comment on utility-scale economics, but, at personal scales, it is, without exaggeration, far and away the bet financial investment you can make today.
Add up everything from the project cost through the tax rebates and utility incentives and ongoing maintenance, here in Arizona, a 5 kW PV plus hot water will offset about 10 MWH annually and pay itself back in about seven years. If you remember the rule of 70, that’s a 10% annual rate of return, and it’s literally “safe as houses,” the recent bubble notwithstanding.
Even if you don’t have the capital to finance it yourself, get a bank loan at 3% – 5% depending on your credit, and you’re left with an investment that earns 5% – 7% initially, and 10% after the loan is paid off.
Even better, it’s not just inflation-proof, it’s energy-inflation-proof. If energy prices were to take off at 10% inflation, your solar-provided energy would literally be as cheap as it is today.
Different regions have different amounts of insolation and different incentives, but I’d have a hard time believing that anywhere in the country, Seattle included, could work out to worse than 4% ROI. And if that’s good enough for a bank to charge for a 30-year mortgage, why shouldn’t it be good enough for you for a 30-year personal solar array?
(A Libertarian of the Rand school at this point will likely complain that I’m parasitizing the government and the utility through the incentives, but I’d respond that both are getting excellent returns on their own investments. The government doesn’t have to deal with the pollution and political fallout from my energy use, and the utility actually makes money off me because they buy peak energy from me at off-peak wholesale rates. Win-win-win, as they say.)
Cheers,
b&
Here’s an interesting page from the NPS on the history and installation of awnings to block sun and rain: http://www.nps.gov/hps/tps/briefs/brief44.htm
I’m really enjoying your work here Tom.
Having now built a half-dozen houses that are quite habitable with minimal fuel input by digging the North side of the structure into the ground (a hillside is best, but dirt-berming is an acceptable substitute), and orienting the South side for maximum thermal gain in the winter, I wonder if you plan to discuss the merits of incorporating tapping into the geothermal constant.
Here in Western Colorado the constant temperature six or seven feet below ground is about 55 deg.
I’ve found that by judiciously juggling thermal contact with that 55 or so degrees, coupled with solar gain stored in interior mass (part of the architecture) it’s possible to have a home that seldom, if ever, drops below 60 degrees without the application of any additional energy at all. And this even with nighttime minimums in the negative double digits.
Climbing from that baseline up to 70 degrees is not such a steep hill at all, for those who just can’t stand to wear a sweater.
Such a strategy cannot be retrofitted, for sure, but as a conscious plan for future homes, modified on a site specific basis, it’s a powerful concept.
That stuff sounds great; also see ground-based heat pumps, for better electricity-based heating and cooling. OTOH, a growing majority of American or even people worldwide are urban, a lot of whom are living in apartments, and at any rate with buildings facing in all four directions, not just south. To make this standard practice you’d need to retrofit or rebuild not just buildings, but whole cities. Doesn’t scale well…
None of those buildings will last forever. In modern cities virtually nothing is built to last for more than 50 years. You just need to start with new buildings and over a long enough time scale everything will get replaced. I don’t think anyone thinks we can just wholesale replace existing structures.
In my neighborhood in Chicago (west of Loyola University), ≈¾ of residential buildings are ≈100 yr old. Densely packed (neighborhood layout in pre-automibile days). Nice buildings, little teardown activity. Great area to live, but not ideal for local passive solar (although a restaurant down the block claims to be the greenest in North America)…
Besides David’s point, I was bringing up the question of urban *design*. A suburban or rural house in Colorado can be built to order in a vacuum. When you replace a west-facing urban home, are you doing to try to make it south-facing? How about an apartment building? What about being shaded by other buildings?
Yay! The human race is saved 🙂
Seriously, is there some downside here that I’m missing?
Doesn’t immediately help transportation; geographically variable; retrofit for passive heating impractical/impossible; intermittency problem not gone, just could be made better than PV/wind. On the whole, I like it, but I’m not jumping up and down.
Care to comment on this style of collector?
http://en.wikipedia.org/wiki/Solar_updraft_tower
[content stripped out by moderator: note these links are about 3 years old]
http://www.foxnews.com/politics/2009/03/21/feinstein-dont-spoil-desert-solar-panels/
AP Saturday, March 21, 2009
===================================================================================================
Environmental Concerns Threaten Solar Power Expansion in California Desert
Saturday, April 18, 2009
http://www.foxnews.com/story/0,2933,517053,00.html
[edited]
The fact that [the stories] are 3 years old is not very relevant. The issues have not been resolved, and they remain active issues. The point is that using large quantities of desert land is not without problems.
One issue within Professor Murphy’s bailiwick is dry vs wet cooling and how the limited availability of water in the US desert will impact solar thermal concepts.
Indirect dry cooling (AKA the Heller system) reduces water consumption in solar thermal plants by over 95% with little performance and cost impact (~5% vs wet cooling). It’s a proven technique that’s used today in gas-fired CC plants and at least one nuclear plant. Nuclear power also benefits greatly from this.
Tom,
As you point out, solar is abundant. It is also intermittent, in predictable and random ways, and about one kW/m2 power density at a good moment. Compared to burning something, basking in the sun is like drinking from a fog. Lower power density implies to larger structures; hence greater embodied energies.
And I recall your note that a nuclear plant is surprisingly compact. This suggests that our sustainable, fourth-generation reactors would be a better resource investment than solar thermal, especially for electrifying our power-dense cities.
Well it occasionally takes some squinting to call nuclear ‘compact.’ For example, the North Anna 2 GW nuclear plant likely supplying the electrons for this comment had a 53km^2 lake built solely for its cooling needs. For comparison, the lake area idly receives 53 GW for 3-6 peak hours a day. Then there is the plant area, the security keep out zone, the low population zone, the U mines, the U enrichment facilities …
http://en.wikipedia.org/wiki/North_Anna_Nuclear_Generating_Station
A nuclear power plant converts thermal energy into electrical energy, and so does the solar-thermal-electric plant discussed. Cooling is requirements are determined by the heat source characteristics. Cooling requirement for an equivalent solar-thermal-electric plant will be greater than for nuclear, since the latter operates at a higher source temperature, resulting in greater Carnot efficiency. So, just to reject the thermal waste heat for solar requires more stuff.
For a thermal power plant to work, one must somehow establish a temperature gradient to extract mechanical energy from thermal. The heat source is only part of the solution; a heat sink is also required. Some sites provide better heat sinks than others. Poor heat sinks, such as desert air or a small stream require cooling towers or lakes, which can dwarf a any power plant. Cooling towers and lakes look big on nuclear sites precisely because nuclear is a wonderfully efficient heat source. As Tom correctly pointed out in a previous post on nuclear, sites taking advantage of an ocean or deep lake for cooling are compact indeed.
BTW, here is a link to a page describing cooling requirements for solar-thermal-electric facilities:
http://www.basinandrangewatch.org/Water.html
Add thermal storage to the mix, and source temperature decreases, and cooling requirements per Joule-electric increase.
A couple responses:
1. I don’t know if solar thermal has an industry standard yet for operating temperature; Tom uses 400C in his article above. On the other hand, nuclear PWR runs the primary loop at ~315C, BWR at 285C. Perhaps next generation nuclear w/ molten salts will run a Brayton cycle and obtain better efficiency, but for the moment nuclear has no Carnot advantage over solar thermal.
2. My original point was not say that solar or wind were compact, but to ask for some reexamination of the claim nuclear necessarily is, at least as built now under NRC, and hence my reference to the 53 km^2 North Anna lake. Even ocean side reactors are subject to keep out zones and the three mile low population zones. Take a look a Diablo – there’s hardly a house or farm for miles, no major cities for 20-30 miles. Same goes for Calvert Cliffs on the Chesapeake Bay and so on.
http://g.co/maps/msmmh
http://g.co/maps/kb43n
3. Solar PV of course requires no water for a cooling cycle.
Falstaff,
Regarding nuclear facility area, your examples are not necessarily exemplary. To pick another random example, the plant I am most familiar with is just north of Two Rivers, WI. It is a 1000 MWe facility, and crops grow within 1000 feet of the containment building, as a quick look on Google maps will confirm. The facility itself is roughly 500 by 1000 feet, including parking lot, switchyard, spent fuel storage, and museum.
Theoretically, logging of the forest could be performed right up to the parking lot. The public is not excluded from the site. The Point Beach Energy Center on site, and you are welcome to visit.
I’ll concede that perhaps one must include the energy storage-due-to-intermittence consideration to get a lower source temperature for solar-thermal, since the maximum source temperature is really determined by material choice.
I’m not sure what “I’ll concede that perhaps one must include the energy storage-due-to-intermittence consideration to get a lower source temperature for solar-thermal” means, but the temperature limit for parabolic trough collectors is about 400 C because the oil used in the pipes begins to break down at higher temperatures. Power tower designs like Gemasolar (http://www.dailymail.co.uk/sciencetech/article-1393879/Gemasolar-Power-Plant-The-worlds-solar-power-station-generates-electricity-NIGHT.html) heat up to 900 C with molten salt storage and achieve 24 hour/day operation during summer months. Designs that reach 1000 C are being tested (http://www.gizmag.com/waterless-solar-thermal-tower-power-plant/16738/ — happens to not use water, either).
So, given that nuclear plants actually operate at *lower* temperatures, I assume you’ll amend your earlier comment to “Cooling requirement for an equivalent nuclear plant will be greater than for solar-thermal, since the latter operates at a higher source temperature, resulting in greater Carnot efficiency. So, just to reject the thermal waste heat for nuclear requires more stuff.”
“Compactness” seems like an odd metric to me. “Cost” (which would include the cost of the land required as well as that of the material required) makes more sense.
If you’re worried about land usage then compact linear fresnel actually uses about half the land of parabolic trough and because the “mirrors” (usually polished aluminium) are flat they’re cheaper to make, too. The key insight is that rather than having a rotating mirror dedicated to each tube you can instead suspend a fixed array of tubes over the collection field and *change* which tube a given mirror is deflecting to according to the sun angle. This allows the mirrors to maintain shallow angles, reducing self-shadowing, allowing nearly all the light in the collection field to be collected (~80%).
You might also want to look up how much land has already been disturbed by coal mining, which is also typically in much higher-value places than the desirable locations for a solar thermal plant *and* has a bigger impact on the land.
Yes, cost would be a better metric. I’d love to see an honest to goodness, apples to apples, total cost comparison of fourth generation nuclear to the latest iteration of solar for generating base load electricity. Doing this will require building them both with open accounting books.
For now, if we look at the compactness of the facility, the amount of earth disturbed, and fuel availability, nuclear seems to come out way ahead of both solar and hydrocarbon.
Rather than spiral off into a nuclear discussion (had plenty on the nuclear post), I will terminate this thread here—perhaps a little late.
Again, why look at the compactness of the facility? If we were short of land then it might make sense, but then it would automatically be included in the cost of the land. The fact is that land happens to be cheap in the places most suited for solar thermal and there’s enough land in the deserts of the world for it not to even be remotely considered a constraint. If you’re really that worried about land use, use compact linear fresnel — that doesn’t suffer from self-shadowing and can collect 80% of the light falling on an area, resulting in half the area for the same power output as parabolic troughs use.
Using an existing parabolic trough system like Nevada Solar One as a benchmark (134 GWh/year, 1.6km2) you could generate the entire planet’s current annual energy consumption (not just electricity) using just 17% of the Sahara desert. The trick is figuring out how to economically get that energy into the forms we want, when and where we want it, not finding space to generate it in the first place.
And the amount of earth disturbed? Given that we have already disturbed, by coal mining, an area equal to that required to provide all power using solar thermal, it seems like we don’t care very much about the amount of earth disturbed. And not only is the degree to which the earth is disturbed by coal mining far greater than simply putting it in the shade, coal also usually happens to be located in much higher-value areas than solar thermal plants would be.
Likewise for water. Even your own link says that dry cooling only adds 10% to the cost of producing electricity. If water is a constraint, use a Heller system; if it’s not, use wet cooling and save some money. Given the uncertainties, 10% is lost in the noise.
Regarding the feasibility of Passivhaus buildings and Solar PV in the US/Canada, it might be worth noting that Berlin and London are at the same latitude as South Hudson Bay. Although they are warmer due to the Gulf Stream, they don’t get any more sun.
Tom,
How much molten salt would we need for your 7 day reserve?
According to the properties I found at http://www.engineeringtoolbox.com/sensible-heat-storage-d_1217.html (2260 kJ/m³/K), and allowing the salt to swing from 140°C to 540°C, I calculate that my 336 TWh 7-day U.S. requirement would take a cubic kilometer of molten salt. Pretty similar in volume to the lead acid battery. No resource pinch in this case. Just a bunch of scary lava-hot goop.
According to http://www.scientificamerican.com/article.cfm?id=how-to-use-solar-energy-at-night and http://www.sciencedirect.com/science/article/pii/S0360544203001932 it costs US$30-50 to install, although apparently “it doesn’t add much to the cost of the resulting electricity because it allows the turbines to be generating for longer periods and those costs can be spread out over more hours of electricity production”.
Using those figures your 336 TWh would cost about 15 trillion dollars. 🙂
I think molten salt is more for short-term energy storage (i.e. to shift electricity production into the night, and possibly get through a cloudy day) rather than long-term. Pumped hydro is much better for long term storage but the problem is the resource is limited (although Scandinavia’s resources can provide an impressive amount of storage for Europe).
Another alternative is making a hybrid solar-natural gas plant, since both can share the back-end and the solar part effectively reduces the gas required, making it more efficient and thereby buying us some time.
Oh, and over-sizing the collector field of a solar thermal plant by a factor of two or three is another useful technique for squeezing more hours out of a day and increasing the utilisation of the generating capacity. At peak periods some of the mirrors are turned away but in return peak generator output can be maintained at lower sun angles. The Gemasolar CSP plant uses both molten salt and an over-sized collection field to achieve a capacity factor of 63.1% and 24 hours/day power output for many months per year. (http://theenergycollective.com/nathan-wilson/58791/20mw-gemasolar-plant-elegant-pricey) At $33,000/kW_avg it’s pretty expensive, though.
“Scandinavia’s resources can provide an impressive amount of storage for Europe”
From http://www.iset.uni-kassel.de/abt/w3-w/projekte/LowCostEuropElSup_revised_for_AKE_2006.pdf, the storage capacity of NORDEL + UCTE is 177 TWh, enough for more than a month of average consumption in EU + Norway combined. So Europeans have no excuse not to use intermittent renewables. 🙂
It’s also interesting because it shows they have already achieved over half the storage capacity that you dreamed of for the US without any fanfare.
Hi Tom,
Despite the fact that I don’t think 7 days is the right figure, for the sake of consistency I think you should include the thermal to ‘useful’ conversion efficiency in the above. 1km^3 was the figure I got for 336TWH of thermal energy with the temp range you show (1.3 actually, but whatever). For 336TWH of electrical energy available to be sent where needed (which is what batteries would allow) you’d need more like 7km^3 at 20% thermal to electrical conversion efficiency (which seems about reasonable with an average thermal gradient of only 320degC).
Like you say, not impossible, but one big lake of salt!
Excellent catch. Comment replies are often done with less care than posts (though I make mistakes in both).
Thanx for the reply Tom.
At a density of 1,733 kg/m3 that works out to about 12 Billion tonnes of salt. When you consider we mine similar to that amount of coal every year it is doable. But not going to be cheap/compact either. BTW present USA salt production is on the order of 50 million tonnes, most of it industrial grade Chloride salts.
We are talking on the order of Powell lake in terms of molten Salt. Having this much molten salt in the desert might cause some serious contamination questions.
WRT cost the IEA has done numerous assessments on CSP that show that with larger scale and technology improvements, the prices can rival PV at the 5 cents range by 2030-2050 range. Nevada Solar One without storage came in at $266 Million for 65 MWp.
Even if 7 days is the right figure, the low energy density of hot salt relative to chemical energy storage means it likely be used for short term high repetition rate, say one day, so back to 1km^3. That is about 11 US Strategic Petroleum Reserves of volume (90 million m^3 each).
For the other 6 days that see more infrequent use, to my mind the production of chemical storage (methane, hydrogen, ammonia, whatever) is favorable.
Iron oxides are nice because they can be piled outdoors. Driving the oxygen off requires two-axis solar concentration —
2 Fe3O4 —> 6 FeO + O2
More at http://www.stobbe.com/library/hydrogen/43-53%20Steinfeld%20Ferrite%20Cycles.pdf
Dojomouse,
“Like you say, not impossible, but one big lake of salt!”
It wouldn’t be necessary to store all the thermal energy in molten salt. Molten salt is needed only to TRANSPORT thermal energy, from the collector to some other large thermal mass. The thermal mass can be made out of any material with a high heat capacity, even sand ground to a fine powder.
This idea is used in molten salt thermocline storage systems. In those storage systems, there is a small amount of molten salt which just transfers the thermal energy to a large thermal mass made out of fine sand.
Google for “molten salt thermocline”. It’s a new idea which is just being implemented now. See, for instance: http://www.nrel.gov/csp/troughnet/pdfs/jpacheco_thermocline.pdf
In my semi-informed opinion, this new idea is one of the most important advancements in renewable energy in the last few years. It was a mistake to assume that the entire thermal mass must be molten salt. Avoiding this mistake liberates us even from the necessity of mining and transporting a large amount of salt. Instead we could just gather sand from the _immediate area_, grind it, and put it in a large storage tank.
I’m certain we have more than 7 km^3 of sand for the entire US. (Of course I have not adjusted here for the differing heat capacities of sand vs molten salt, and have not adjusted for the increased volume of unpulverized sand which has big air gaps between the grains of sand. Obviously 7 km^3 is a rough estimate, but the point still stands that we are not running out of material which could serve as a thermal mass).
Tom M – Thanks; and yeah, when you crunch as many numbers as you do there is bound to be the occasional slip!
Tom S – that’s an interesting point; I hadn’t really considered that the main benefit of salt was as a heat exchange medium rather than a storage medium itself. Certainly other substances are cheaper and easier to insulate. The approach you mention makes sense – don’t know if it’s yet the biggest game changer for renewable energy but certainly interesting.
Falstaff – I totally agree. High cost, high efficiency storage only makes sense with high cycling frequency. For longer duration storage (which is only occasionally deployed) a low $/kW solution is far preferable… and the tolerable penalty in efficiency is actually very high. My point was just that IF you wanted 7 days the volumes would be yadda yadda.
Of course, if we could find LOW cost high efficiency storage then we’d be sorted, but for now my sense (like yours) is that chemical storage is the way to go… especially since my vote is pretty much with solar (subvote nuclear) these days and there are seasonal variations to worry about which require bulk storage at low cost with low ‘self discharge’.
I don’t understand why those parabolic trough collectors have a focal point in the air. Wouldn’t it be better to have a pipe running along a small trench in the ground and have half-parabolas looming over them (each twice the area of the two halves of the original parabola: no change in size, and no issues I can see with the change in shape)
That way the pipes would be shielded from the wind, well insulated by the ground, and mechanically supported using less construction material. Also, the half-parabloas can cover them over at night.
Since reflectors bounce, and the sun is “up,” the reflector generically wants to be below the focal point. Lenses (perhaps Fresnel) would allow your scheme. Or the extreme-sloped portions of the parabolic reflector (which is probably what you are thinking)—and that’s harder to pull off. There would necessarily be a lot of wasted space between the parabolic side-segments, and the tilt of the side segments would be very steep so that the projected collecting area is small compared to the length (expense) of the reflector. The cost/benefit sides toward the bowl under the pipe, I believe.
Two reflections are way better than one, including for having the many kilosuns striking down on a target: as you say, the first bounce is up, but the second is down. Diagrams in “Panorama of dual-mirror aplanats for maximum concentration”, Gordon, Ostroumov, Feuermann … library computer is running out of time, so I hope that’s enough to find you it.
I remember back at college (Va Tech), we had a school wide heating system that provided hot water to radiators throughout the buildings on campus. This got me thinking about how efficient it would be to use the heat from a solar collection system directly to heat home & water, instead of turning it into electricity.
Do you have any thoughts, calculations or links for comparing the two methods for distributing energy for household heating needs?
Thanks,
Russ
Thanks Tom, this post pretty much confirms my conviction towards building a passive solar home on my property, (to replace a simply horrid 36 year old ‘mobile home’). A challenge I had not expected was the difficulty in finding a licensed architect that knows how to design one. This doesn’t seem to be something that has ever received much attention in architectural schools, and building codes in some areas have only recently started to accommodate them. I expect most building inspectors wouldn’t know what to make of them when they saw them. They tend to be a tradition oriented lot that don’t like their jobs being complicated by new things.
Secondly, it seems every endeavor has its unexpected consequences. I’m sorry I can’t find the link to it now, but I recall reading a few years ago about a solar thermal plant in Spain that was fighting for profitability because of an unexpected consequence. The concentrated light flowing up towards the tower also heated the air it passed through. This caused considerable air thermals to rise above the plant that attracted thousands of birds every day. The birds would then leave their droppings on the mirrors, necessitating a veritable army of cleanup crew to clean the mirrors every day. this raised the labor cost so far above expectations that the plant was loosing money on their fixed price energy delivery contracts. The plant operators were considering options like shooting the birds. I wonder what the unexpected consequences of that would be?
After thirty something years of construction and farm work out in the sun, I‘m with you on the abundant. I always wondered why they’d say it was ninety five or a hundred degrees in the shade. What was it out in the sun where I was all day? When I’d look for some relief it was easy. Go to the shade. Shade’s a life saver.
What would be the dollar value of blocking the heat gain to all the air conditioned buildings from solar radiation.
It has been reported that reflective roofs and pavements “may be the single most cost effective energy and climate strategy” by climateprogress.org http://thinkprogress.org/romm/2010/07/21/206463/cool-roofs-energy-global-warming/
One step better than a reflective coatings is to block the solar radiation with green leaves. The leaves will suck up some CO2 and store some energy in chemical bonds instead of reflecting the energy back to heat the atmosphere.
I’ve got some shade projects including grape vines growing on my roof on support wires. There are photos on solarincome.com. http://solarincome.com/GreenShade_Systems/HOME.html
Seems like since A/C came around people don’t know what shade is. My bet is that in the not to distant future they’re going to find out.
Thank you for an excellent article, Basking in the Sun. During the 70’s the firm I worked with got a grant to explore solar ponds. The setup was super simple; an insulated hole in the ground with a membrane to control evaporation. Alas the membrane was a silicone oil that turned the pond into a death trap for birds and insects. The thought keeps nagging at me…what if we had used bubble wrap? The ponds were cheap enough and the heat was low grade but lots of it. Could you comment on solar ponds?
What do you think about this:
http://www.dlsc.ca/how.htm
Is there any potential to scale this down?
Wonderfully elegant storage design for space and water heating from solar that works with little assistance even in the high latitudes, over entire seasonal timelines. I see it was -15degC up in that Alberta neighborhood yesterday w/ 40degC heating water entering homes.
A take away point here is the storage of low quality energy, i.e. 40-90degC underground water, is technically trivial in comparison to electricity storage (or pumped hydro). In the US, some 50% of residential and 30% of commercial energy is space and water heating/cooling according to Wiki. No doubt some small fractional of industrial processes require only low quality energy. The calculation for the future national battery (storage), 2 TW for 7 days or 336TWh, would be more useful I think if it set aside space/water heating/cooling, perhaps not for the near term but at least for the more distant, theoretically solar powered future.
“Wonderfully elegant” maybe, but you should also add “uneccessarily complex and very expensive”.
I was personally involved (I wrote the water efficiency standards), with this property development (52 homes and a solar collection and geothermal storeage-retrieval system) and it is great, until you look at what it cost to build.
It was about a $5m project (back in 2002) or roughly $100k per house. Now, there were some high initial costs in there, for designing something that hadn’t been designed before, but the construction costs – lots of pipe and boreholes only get worse with time.
Also, there is not much economy of scale for the reservoir – there is less heat loss from the square-cube rule, but you still need just as many boreholes per 1000 cu.m of storage medium. If you want to see a rapidly inflating cost, get a quote for drilling…
There are other complications too. The system is operated as an energy utility, the heat coming back to each house is metered, and people billed accordingly. So there is extra overhead and expense, plus the o&m of the pumps, meters etc.
It would have been far more elegant, simple, and cheap, to just put the “storage medium” directly under each house – (dig out a bit extra for the basement and put in insulated dry sand) where the heat loss goes into the house and there is no utility involved.
Not only that, the seasonal storage here is really not necessary – that location gets 2000+hrs of sun a year (more then Miami) and typically has very sunny and clear winters.
You could spend half the $100k per house building it close to Passive House standard, and then you could easily collect the remaining winter heat – in winter, and dispense with the storage entirely.
The project is deemed a “technical success” because it works, but it is an “economic failure” because it is so expensive that not another one has been built or even planned in the *ten years* since.
This was a complex answer to a simple problem. Keep it simple, and affordable, and it is much more likely to actually get used widely
My immediate reaction to the idea of solar thermal for electricity generation was that it would quickly run into problems with fresh water availability for the cooling towers. Places like the Middle East could avoid this problem by placing their solar plants by the seas, but those are politically volatile places and I think it would be best to try to avoid relying on them for energy. In North America, the best sites for collecting solar energy are not near large bodies of water.
Above it was mentioned by someone that water consumption for the cooling function can be greatly reduced by the Heller system of dry cooling with limited effect on performance. I don’t know much about this. Tom, do you have an opinion? The second law dictates that to produce electricity at say 30% efficiency in the cycle, the other 70% must be dumped as heat, most of it through the cooling system. I find it hard to believe that dumping this amount of heat in a baking hot desert without the use of latent heat absorption would be possible without a significant hit to thermal efficiency.
I agree with the first paragraph. For solar electric to have a future via thermal conversion, it has to find a way to a high temperature Brayton cycle with out water. Either that or PV wins the day. As I recall there seem to be some hints of this already by way of solar thermal – natural gas hybrid plants, where solar heat is used to boost gas temperature.
Just in quick numbers, if a square kilometer of land is producing 40 MW of electricity in full sun, then roughly 100 MW is going into heat. Imagining a collector along one side of the square kilometer soaking up a 4 m high column of air streaming through at a modest 2.5 m/s gives me 10,000 cubic meters (12,000 kg) of air each second. At a specific heat capacity of 1000 J/kg/C, I will raise the temperature of the dry air by about 8°C. So not a trivial heat increase, but not alarming, either. My numbers are easily changed to reduce this further (pull air in faster, collect more of it, etc.).
Another perspective is that without the solar thermal plant, the land would be absorbing roughly the same amount of thermal energy anyway, which is why the desert is hot to begin with! Now that land is partially shaded, but the collectors are filling the gap (and then a bit more).
“The low-tech nature of solar thermal makes it especially robust in tough times.”
I think you are aliasing two different advantages here. One, it is lowtech, and can be improvised and cobbled together. Two, is scales. It is not just the skill level required, but also the amount of manual labor to get above the threshold of having a working device. I can build a simple device in my backyard, but the same principles work on an industrial scale. Hydro (dams, mills) has a similar scalability, and while it also has tight location constraints (liquid water, elevation difference) it had many hundreds of years of use throughout civilization (though not for electricity or heating).
That brings me back to robustness. Solar thermal (e.g. for heating) has less, and it would be interesting to find out why, even setting aside that electricity was not exactly a priority hundreds of year back. Mirrors and lenses? Heat vs. power? If we could convert focused heat into piston movement efficiently and simply, could we build a simple “solar mill”?
“If we could convert focused heat into piston movement efficiently and simply, could we build a simple “solar mill”?”
Ahh, the Stirling Engine. I admit I don’t know much about them, other than that they have never been mass produced. Maybe that will change soon?
http://www.stirlingengine.co.uk/ks90-black-ltd-30-p.asp
http://www.stirlingengine.com/product/78
Building for solar heating — or cooling, with air movements — has an old history in many cultures; we’ve kind of lost a lot of techniques, between air conditioning or urban cramming or cheap housing or all of the above. Solar lighting, solar drying of raisins, meat, or laundry
http://en.wikipedia.org/wiki/Solar_energy#Applications_of_solar_technology
For mills… you need a combination of concentrable direct solar power, and good mills that can be turned by hot air, and given that the Industrial Revolution led in England, Europe, and New England, industrially useful direct sunlight isn’t a feature of such places. And then, cheap fossil fuels!
OTOH, solar hot water seems to have been an overlooked application in history.
http://www.solarhotwater-systems.com/the-solar-hot-water-heater-a-brief-trip-through-time/ claims a few older examples.
Hi, love your blog, but Im concerned about the simplicity of you coverage of the solal thermal electricity. We all know its abundant, but it’s the economics and the energy return on investment (EROEI) that really is interesting. Solar, compared to coal and gas etc comes in pretty low concentrations and energy quality. (See http://en.wikipedia.org/wiki/Energy_quality and notice that in Odums table solar energy comes dead last).
To use solar energy for the same tasks we generally use much higher concentration/energy quality sources like coal means the economic and eroei factors at play in concentrating it are more the issue than its initial abundance. Your 15% efficiency will likely be much lower when all costs of construction, keeping glass clean and undamaged and maintaining tracking systems, grid and intermittency problems are integrated into it. And the current €0.23–0.15/kwh cost reflects this. Its an old technology as well, so all the current clams of breakthroughs around the corner might be a bit like your fusion example…
I think what Tom is doing with his blog is showing us the true possibilities in a future we simply can’t predict, by using physics that we can.
I cannot tell you how much solar power will cost in the year 2020, but I can tell you the maximum efficiency of a steam turbine. That gives me something to work with.
As we have gone down the various ideas for longterm energy, we have found some candidates that the math says are definite possibilities. But I think the more telling ones are the cases that are simply not practical no matter how you slice it.
We only have so many research dollars to invest, and while economics will ultimately tell us which ideas will get built, the math can quickly tell us which ones to drop off the board altogether.
Im aware of what toms trying to do with this blog abd really appreciate it, I’m just trying to point out that his method of top down calculation of total resources gets less and less worthwhile as you move to less concentrated forms of energy, especially if your not really looking at EROEI or energy quality. A diffuse energy source like solar just isn’t going to power the same society as coal because of the effort to concentrate it. Fossil fuel comes pre-concentrated by millions of years of geological forces. A better question than how much solar energy is there is how large is the gradient between the energy source and its surroundings, and what kind of self organising system can be powered on that difference…
do you know the Four solaire d’Odeillo in France
solar furnace opened in 1970 ( 41 42 years old ! )
http://fr.wikipedia.org/wiki/Four_solaire_d%27Odeillo
Odeillo Font-Romeu ( Olympic winter game village ! )
http://upload.wikimedia.org/wikipedia/commons/9/9a/Font_Romeu_France.jpg
On calculating EROEI
Exactly how far back and which processes are (or should be) included in the Energy Invested calculation for the various energy systems.
For example, in the case of a PV system, does it include the energy required to extract the ores and process the steel which is then transformed into a mechanical excavator which is used to extract the silica which eventually ends up in a furnace for processing into silicon?
How about the energy used to make the specialised machinery and equipment that is used to manufacture the silicon ingots or the equipment used to saw the ingots into wafers or even the energy required to make the equipment that recycles the slurry used in the wafer cutting process?
Is the energy that is used to extract and process oil into fuel that powers the trucks and ships that carry the silicon wafers to the PV cell assembly factory in China counted?
It seems to me that the potential ‘energy steps’ used to produce electricity from a PV cell (and others) are endless. What is and is not counted?
They number of steps is certainly not endless, and it is fairly simple to put a reasonable upper bound on the energy invested. The costs are basically divided into energy and labor. All machines used in the process ultimately come down to energy and labor as well, which is amortized over their lifetime and therefore priced into the final product. Raw materials cost nothing, only the labor and energy required to get them out of the ground does. While solar cells are subsidized by the government, that happens after the point of sale, so the sticker price is pretty much the real cost.
So take the price of the solar cell or thermal collector, see how much coal you get for that, multiply by 3 for carnot efficiency if you’re making electricity, and you have a pretty good guess. Interestingly this also means that once they are economical without subsidies (hidden or otherwise), they also have positive EROEI.
caveats:
– This argument only holds in the absence of significant subsidies before the point of sale, otherwise all bets are off
– I assume labor is a small portion of the cost, which seems fair in an economy where everybody uses orders of magnitude more fossil energy than their own metabolism produces/consumes (and a large part of the cost of labor is actually just energy again, namely the energy required to sustain the human)
Good points Felix, I largely agree. A couple points however.
“Interestingly this also means that once they are economical without subsidies (hidden or otherwise), they also have positive EROEI.”
I don’t know if it’s quite that simple because with renewables the energy return happens over a longer time frame (20 years or so). And how long they produce exergy all depends on how well you maintain them. Defining a cutoff-age for renewables is less clear cut than burning oil once in an engine, after which it is gone forever. With oil by contrast you can get your return on investment in 5 years. So the economics are different, since investors want to see a return sooner rather than later. But society is more interested in total energy return. This is where the government should step in, to provide greater assurances of long term investment security.
I’d argue that labour is 100% of all costs. Every natural resource is free; what we pay for in the price is largely a reflection of how much human labour is required to harvest, transform, and deliver it to the point of sale. Ultimately, all money is a claim on human labour, and therefore ecological productivity.
I think mcn’s concerns about all the factors involved in calculating EROEI for solar are equally if not more applicable to fossil fuels. The entire economic and monetary system is structured around oil; without it the dollar is literally worthless. That’s one reason why the US will never voluntarily transition to a predominantly oil-free infrastructure because this would entail the destruction of the monetary system. The two events are synonymous. Therefore, teasing out what kinds of subsidies fossil fuels get becomes even more difficult, but they are systemic and much greater than most people realize.
note that I was merely suggesting using the _current_ cost of the cheapest fossil fuel as a proxy to estimate the _energy_ embodied in a product. For this point it doesn’t matter how we obtained that primary fuel, or what other economics play into it.
That fuel (coal) – for which we know EROEI is high – just becomes a convenient starting point for this chain which of course ultimately ends with just labor, but if we try to calculate from there we don’t get the number we’re looking for.
And yes, the economics are very different, once you talk about those you would have to factor in interest on the initial investment sum, cost of lost opportunity, and whatnot. Doesn’t change the energy balance though, in fact it just strengthens my point, since it means that once solar is economical without subsides, it’s actually quite a ways past EROEI=1.
And when I’m awake and have had a coffee in the morning, I’ll actually calculate that and see what comes out.
Jeff Vail wrote a great series of essays on EROEI a few years ago that you might find useful, finding that it’s often too complex to accurately calculate, and that professional estimates could be up to an order of magnitude in disagreement. His conclusion was that price, though flawed, is probably our best method of estimation… http://www.jeffvail.net/2009/06/renewables-hump-5-proxy-eroei.html
There have been a number of studies over the years that attempt to calculate the EROEI for solar panels and systems. There is a reasonable snapshot from NREL here.
http://www.nrel.gov/docs/fy05osti/37322.pdf
which indicates 1 year to 4 years energy payback. This is in line with other studies I have seen. Even if it is out by a factor of 2 (unlikley) it is still a pretty good return.
I was just wondering if it might be possible to store solar thermal energy of these
relatively low temperature chemically as Dinitrogenmonoxide, perhaps using Singlet-Oxygen
(also produced by sunlight) as an intermediate/catalyst.Does it react easier with N2 than Triplet-Oxygen? The nitrogen and oxygen could be taken directly from the air, and the N2O might produce heat or even motion energy by decomposition later on.
But my chemical intuition tells me you´d need higher temperatures and many steps to keep the reaction going, and N2O unfortunately is a strong greenhouse gas.
It’s interesting that the responses drifted pretty quickly away from domestic scale solutions to the mega-scale thermal plants even though domestic heating and hot water usage account from 30% to 50% of energy use depending on where you live. Implementing direct solar thermal solutions at this level, including energy efficient design, would make a huge impact on the amount of energy needed from mega scale utilties. But high-tech and big is sexier.
Compare the following conversations
“Tom, check it out, I’m saving the planet!”
“How?”
“4kW of gleaming new PV panels on the roof!”
“Cool”
“Tom, check it out, I’m saving the planet!”
“How?”
“Draft excluder on the front door!”
“What the …?”
and yet, closing a 1 inch gap at the bottom of a door can save you up to 1500W of heating if it’s 10C cooler outside.
The advice I give people who are looking to spend on energy savings is to focus their efforts (and money) on
1. making the house more energy efficient, then
2. investing in solar hot water and then
3. investing in PV.
That gives you the biggest bang for your buck. Of course, local incentives or tax breaks might change the order. But incentives come and go, energy savings are forever (well, a long time at least)